In the rapidly evolving space economy, understanding the fundamentals of rockets and orbital mechanics provides crucial context for investment decisions. This primer walks through the physics and economics of space access, explaining why recent innovations in both rockets and satellites are creating new market opportunities. From the basic principles of propulsion to the strategic advantages of dedicated small launch vehicles, this guide aims to equip investors with the knowledge needed to evaluate opportunities in the space transportation sector.
How Rockets Work: Physics 101
Newton's Third Law in Action
At their core, rockets operate on a remarkably straightforward principle: they move by hurling mass out of their engines. For every action (exhaust gas rushing downward), there's an equal and opposite reaction pushing the rocket upward. In plain terms, a rocket is essentially a flying cannon or rifle that recoils in the opposite direction of its exhaust. If you have ever fired a rifle, or held a garden hose with a nozzle, you have experienced Newton's Third Law.
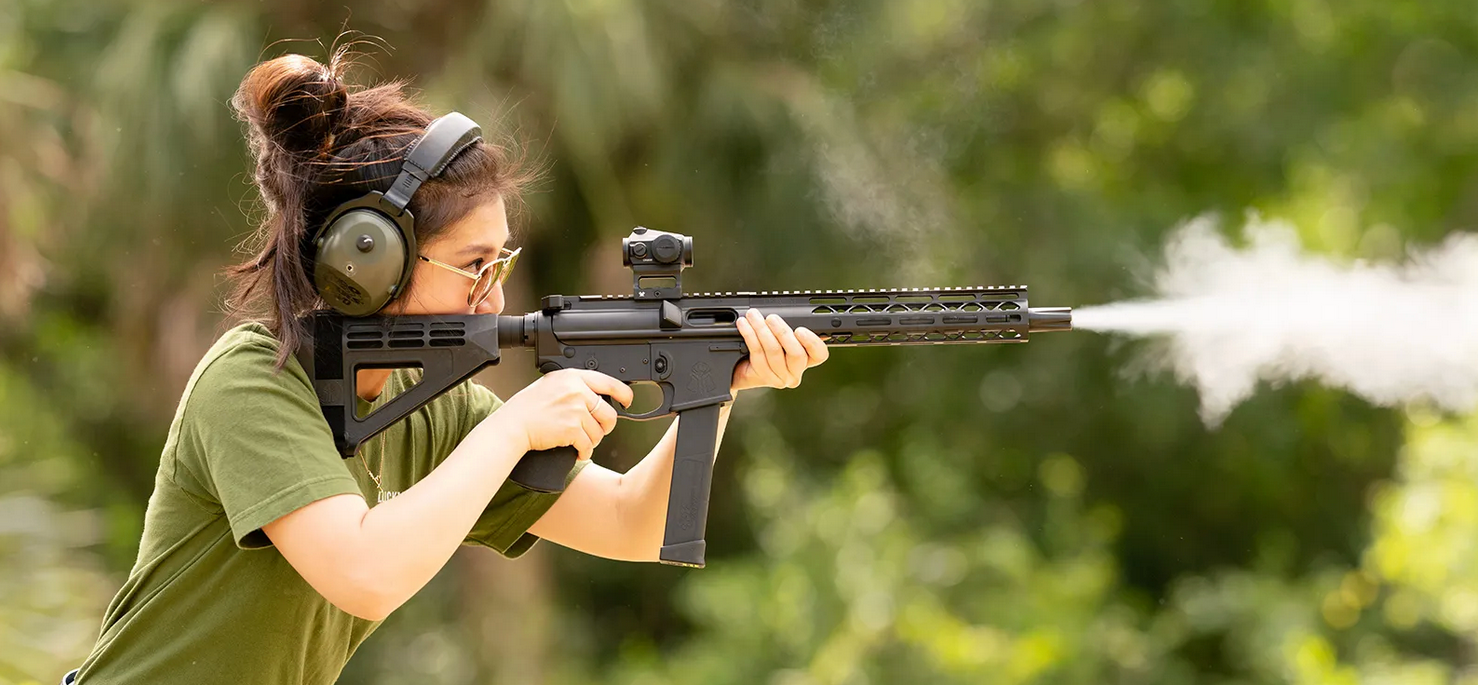
Multi-stage rockets stack several "cannons" in tiers; when the lower stage burns out, it's dropped to shed dead weight, allowing the next stage to fire without carrying empty tanks. This staging is a clever hack to overcome gravity and reach orbit with limited fuel, and reach the required velocity of about 8 times the speed of a rifle bullet.
The Tyranny of the Rocket Equation
Getting to orbit is brutally hard – most of a rocket's mass at liftoff is propellant, not payload. In fact, only about 5% of an orbital rocket's weight is actual payload. The Space Shuttle, for example, was approximately 94% fuel by mass at launch. This extreme fuel-to-mass ratio stems from the rocket equation: every extra kilogram you want to put in orbit demands exponentially more fuel to lift it.
For the Saturn V Moon rocket, each additional kilogram of payload required 23 kg of extra fuel. No wonder rockets are basically giant fuel tanks with an engine at one end and a tiny satellite (or capsule) on top. In fact, if humans had evolved on a planet much larger than the earth, it would be essentially impossible for us to leave it's gravity by using chemically fueled rockets.
Why Rockets Are Mostly Fuel
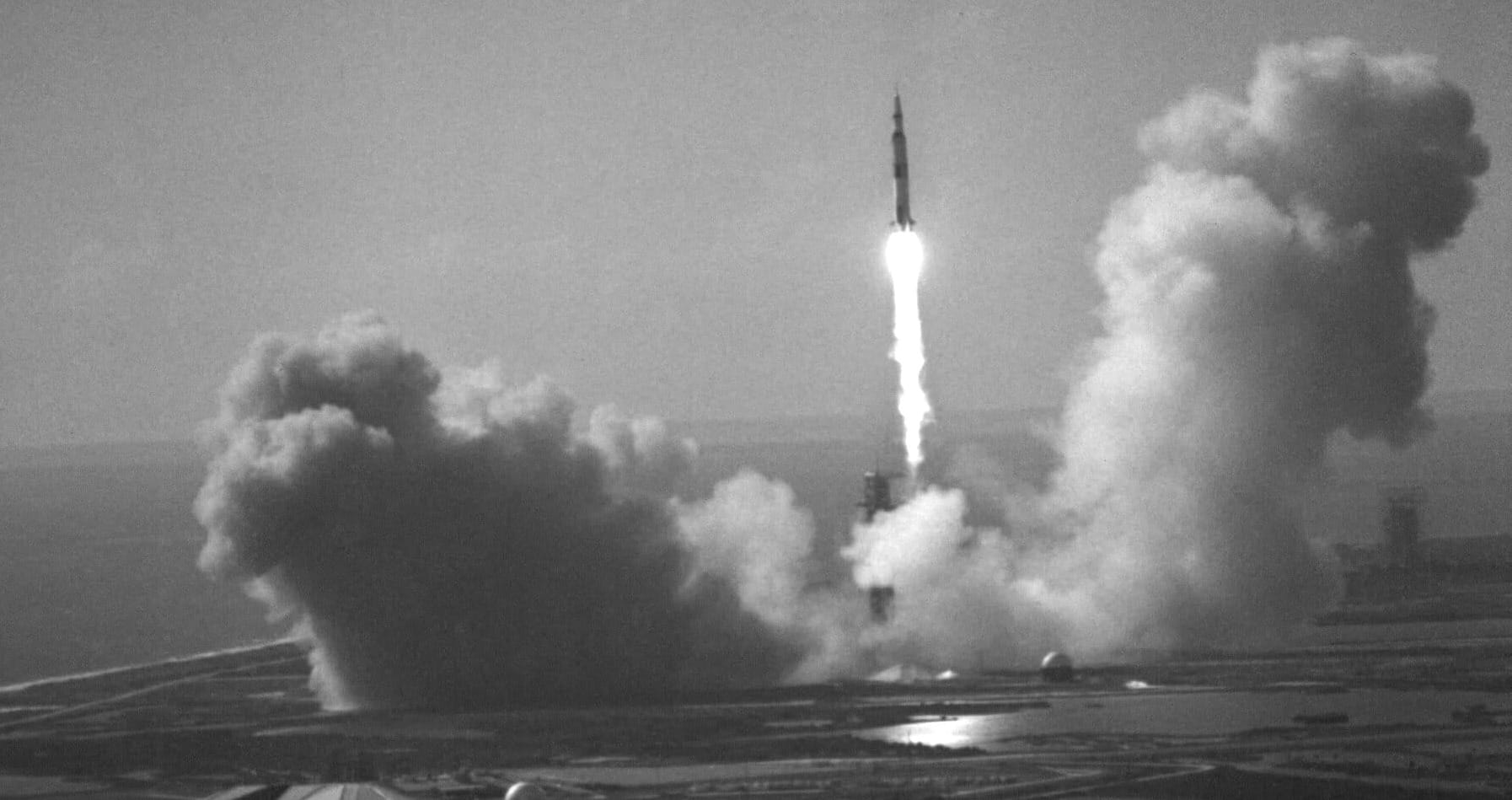
To reach orbital speed (approximately 17,500 mph), a rocket must expend an immense amount of energy. Because chemical fuel has limited energy density, a rocket needs tens or hundreds of tonnes of propellant to deliver just a few tonnes to orbit. Physics leaves little wiggle room – designers shave off every gram of unnecessary mass and use ultra-light, high-strength materials (which are expensive) to maximize the useful load.
The result: a typical launch vehicle is a slender metal balloon of explosive propellant. As one space pundit quipped, rocket engineering is about "solving for the least absurd way to get to orbit."
Design Trade-offs: Cost vs. Lift vs. Reusability
Rocket engineers constantly juggle performance and cost. A larger rocket can lift more but generally costs more to build and launch. Reusability is the modern game-changer – SpaceX proved that flying a booster multiple times can dramatically reduce per-launch costs. By recovering and reusing first stages, SpaceX's Falcon 9 achieved a major cost reduction in orbital launch, upending the industry.
However, reusability comes with design compromises: a reusable booster must reserve fuel for landing and add landing legs, slightly reducing its lift capacity. There's a balance between maximizing performance (expendable rockets) and designing a cheaper ride (reusable ones).
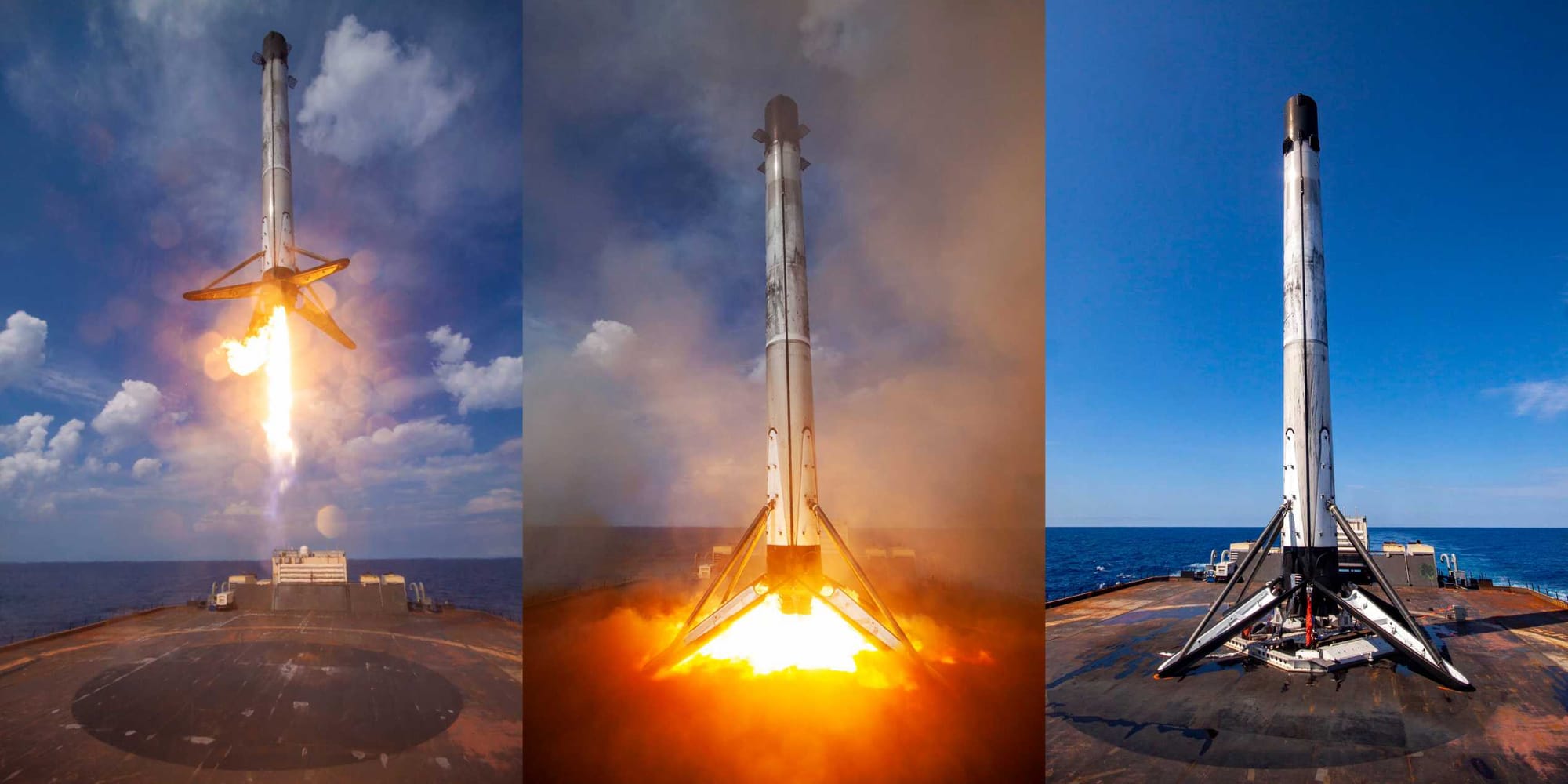
For investors, falling launch costs (thanks to innovations like reusable rockets) are a key enabler of the new space economy – what was once a $10,000+ per-kilogram endeavor is becoming far more affordable, opening the floodgates for launching satellites at scale.
What Is an Orbit, Anyway?
Let's take a step back and explain something fundamental that's easy to overlook: what exactly is an orbit, and where does a rocket go when it leaves our atmosphere?
The Basics: Falling Around Earth
When a rocket launches from Earth, its ultimate goal isn't simply to go "up" into space. Rather, it needs to achieve something more complex - an orbit. An orbit is essentially a balance between two forces: gravity pulling an object toward Earth, and the object's forward momentum trying to carry it away in a straight line.
Think of it this way: if you throw a baseball, it travels in an arc before hitting the ground because gravity pulls it down. If you could throw that baseball fast enough (about 28,000 km/h or 17,500 mph), something remarkable would happen - it would still fall toward Earth due to gravity, but Earth's surface would curve away beneath it at the same rate it falls. The result? The baseball would continuously "fall around" the planet, never hitting the ground. In fact, Sir Isaac Newton imagined this exact thought experiment with the use of a cannon, which he published in Principia.
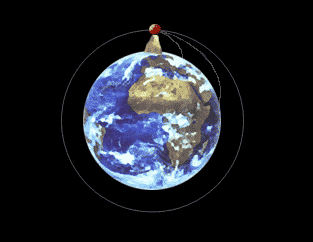
This is exactly what an orbiting satellite does. It's not floating or hovering; it's perpetually falling toward Earth while moving forward so quickly that it misses the ground. Sir Isaac Newton illustrated this concept with his "cannon on a mountain" thought experiment - fire a cannon fast enough, and the cannonball goes into orbit.
Getting to Orbit: The Sideways Challenge
This explains why rockets don't just go straight up. They initially launch vertically to get through the thickest part of the atmosphere, but then they pivot and accelerate horizontally. This maneuver, called a gravity turn, is necessary because reaching space isn't just about altitude - it's primarily about achieving the tremendous sideways velocity needed to stay in orbit.
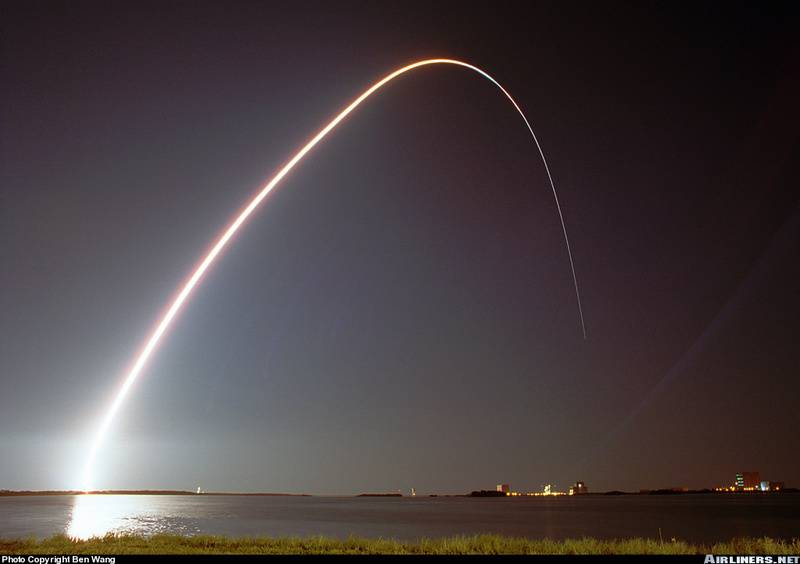
As rocket engineers often say, "Getting to space is just about going up. Staying there is about going sideways really, really fast."
In fact, reaching orbital altitude (around 200-400 kilometers up) requires far less energy than achieving orbital velocity. This is why suborbital tourist flights that just "touch space" before falling back are much easier than missions that actually place something in orbit. The moment the engines turn off, the vehicle begins to fall, which results in the sensation of "weightlessness."
Understanding Orbits (LEO vs MEO vs GEO)
Not all orbits are created equal. Low Earth Orbit (LEO) is close to Earth (typically 300-1,200 km up), Medium Earth Orbit (MEO) is in the mid-range (a few thousand to ~20,000 km), and Geostationary Orbit (GEO) is much higher at 35,786 km above Earth's equator.
In LEO, satellites complete an orbit in about 90 minutes, zipping across the sky relative to ground observers. In GEO, a satellite takes exactly 24 hours to orbit – matching Earth's rotation – so it appears to "hover" over the same spot on Earth's surface. That's why your satellite TV dish points at a fixed position in the sky – it's aimed at a GEO satellite.
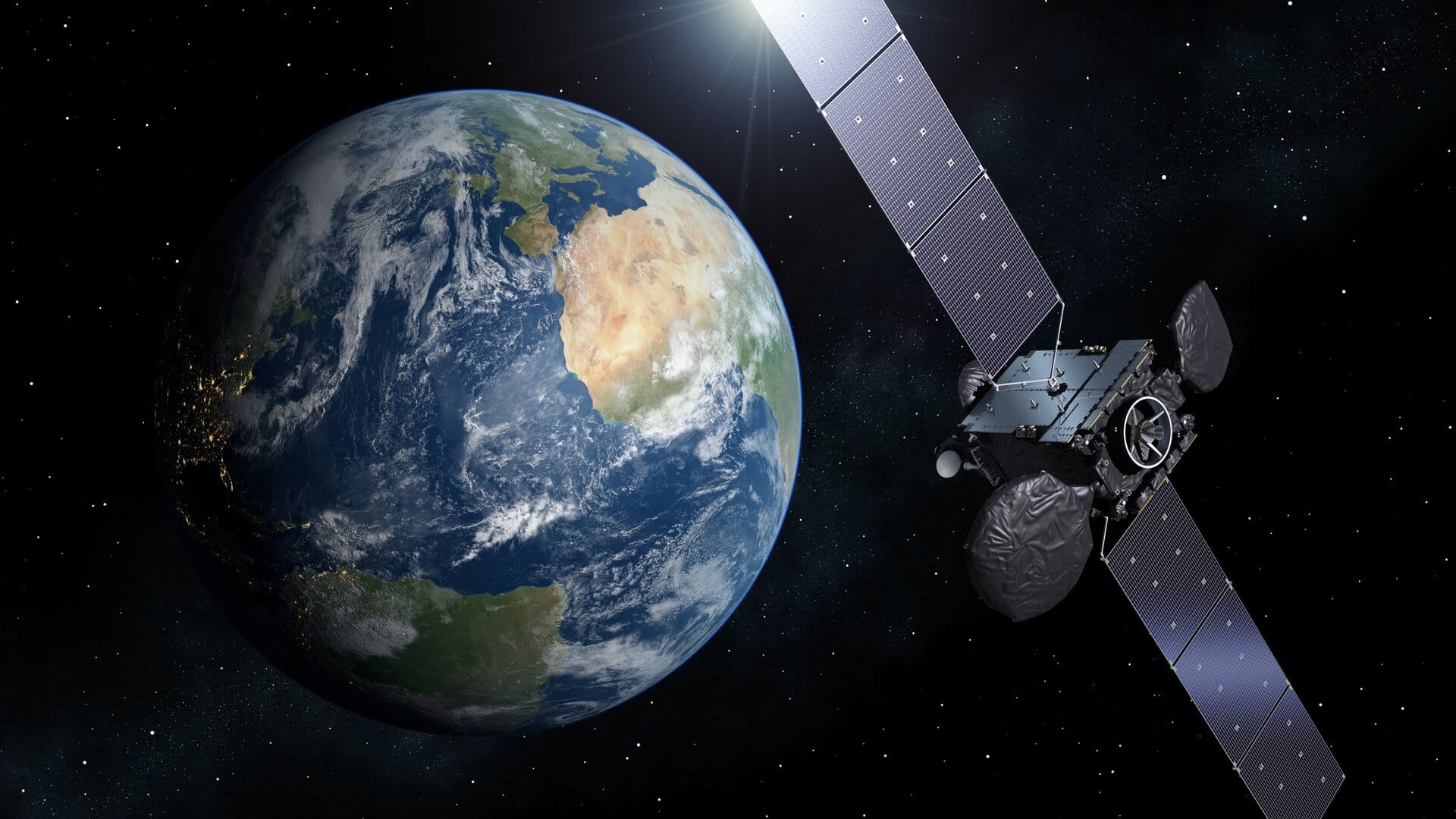
MEO orbits sit between these extremes; for example, GPS satellites orbit at approximately 20,000 km with a 12-hour period. Another specialized orbit is Sun-Synchronous Orbit (SSO), a near-polar LEO where satellites pass over any given latitude at the same local solar time each day – ideal for consistent lighting in Earth images.
Knowing these orbital regimes is essential because altitude and inclination determine a satellite's coverage, ground speed, and communication characteristics.
Where Satellites Live: The Orbital Zone Use-cases
Once in orbit, satellites don't all reside at the same altitude. Instead, they occupy several distinct zones or "neighborhoods" around Earth:
- Low Earth Orbit (LEO): Between roughly 200-2,000 kilometers above Earth's surface. This is where the International Space Station, most Earth observation satellites, and many communication constellations like Starlink operate. Satellites here complete an orbit every 90-120 minutes.
- Medium Earth Orbit (MEO): From 2,000 to about 35,000 kilometers up. This region hosts navigation satellite systems like GPS, GLONASS, and Galileo, typically orbiting at around 20,000 kilometers with a 12-hour period.
- Geostationary Orbit (GEO): Exactly 35,786 kilometers above the equator. This special orbit is where satellites move at precisely the same rate as Earth rotates, allowing them to remain fixed over one spot on the ground. This is ideal for TV broadcasting, weather monitoring, and some communications.
- Highly Elliptical Orbits: Some specialized satellites follow elongated oval paths that bring them close to Earth at some points and very far away at others. These are useful for observing polar regions or providing communications to areas not well-served by geostationary satellites.
Orbital Inclination: Not Just How High, But Where
Beyond altitude, another crucial aspect of an orbit is its inclination - the angle between the orbital plane and Earth's equator. This determines which parts of Earth a satellite passes over:
- Equatorial orbits (0° inclination) circles Earth directly above the equator
- Mid-Inclination orbits (45° inclination) circles Earth at a "slant," between the equator and north-south over the poles
- Polar orbits (near 90° inclination) pass over or near both poles, eventually seeing all of Earth as it rotates beneath
- Sun-synchronous orbits (97-98° inclination) are special polar orbits where satellites pass over any given point at the same local time each day, providing consistent lighting conditions for Earth observation
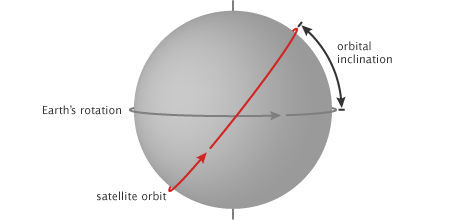
Why Orbits Matter for Investors
Understanding orbits provides essential context for space investment decisions. A satellite's orbit determines:
- What it can see or communicate with on Earth
- How frequently it passes over a given region
- How long it can observe or provide service to a region (and how many satellites are required to cover a region)
- How much power it needs for communications
- What kind of rocket is needed to place it there
A constellation's orbital design directly impacts its service quality, global coverage, and ultimately its commercial viability. Some services require specific orbits to function - GPS wouldn't work from geostationary orbit, and TV broadcasting would be impractical from low Earth orbit.
When evaluating space ventures, investors should consider whether the proposed orbital arrangement matches the business model. Is the company planning to deploy enough satellites at appropriate altitudes and inclinations to deliver its promised service? Does their launch strategy effectively support their orbital deployment needs?
In essence, orbits are the real estate of space - and as with terrestrial property, location matters tremendously.
Speed vs. Altitude: Why Lower = Faster
Satellites in lower orbits must travel faster to avoid being pulled back by gravity. A LEO satellite moves at approximately 7.8 km/s (28,000 km/h), completing an orbit in about 90 minutes. From the ground, it streaks across the sky – the International Space Station (at ~400 km altitude) passes from horizon to horizon in roughly 10 minutes.
By contrast, a GEO satellite at 35,786 km has an orbital speed of about 3 km/s and effectively "stays" above one longitude (because it takes 24 hours to circle Earth). This presents a fundamental trade-off: coverage area versus signal latency.
A single GEO satellite "sees" about one-third of Earth's surface – enormous coverage, which is why just three GEO satellites can blanket most of the globe for communications or weather monitoring. But this distance means signals take noticeable time – approximately 240 milliseconds one-way (~0.5 seconds round-trip). This introduces delay (latency) in communications, noticeable in satellite phone calls or live TV broadcasts.
LEO satellites, being much closer, have significantly lower latency – typically 20-50 milliseconds (comparable to terrestrial fiber links). This makes LEO constellations attractive for real-time internet services and 5G, where a half-second lag from GEO is problematic. However, a LEO satellite's coverage is limited – it might only see a patch of Earth a few thousand kilometers across. And finally, a LEO satellite requires far less radio and amplification power to close a radio link with a mobile device.
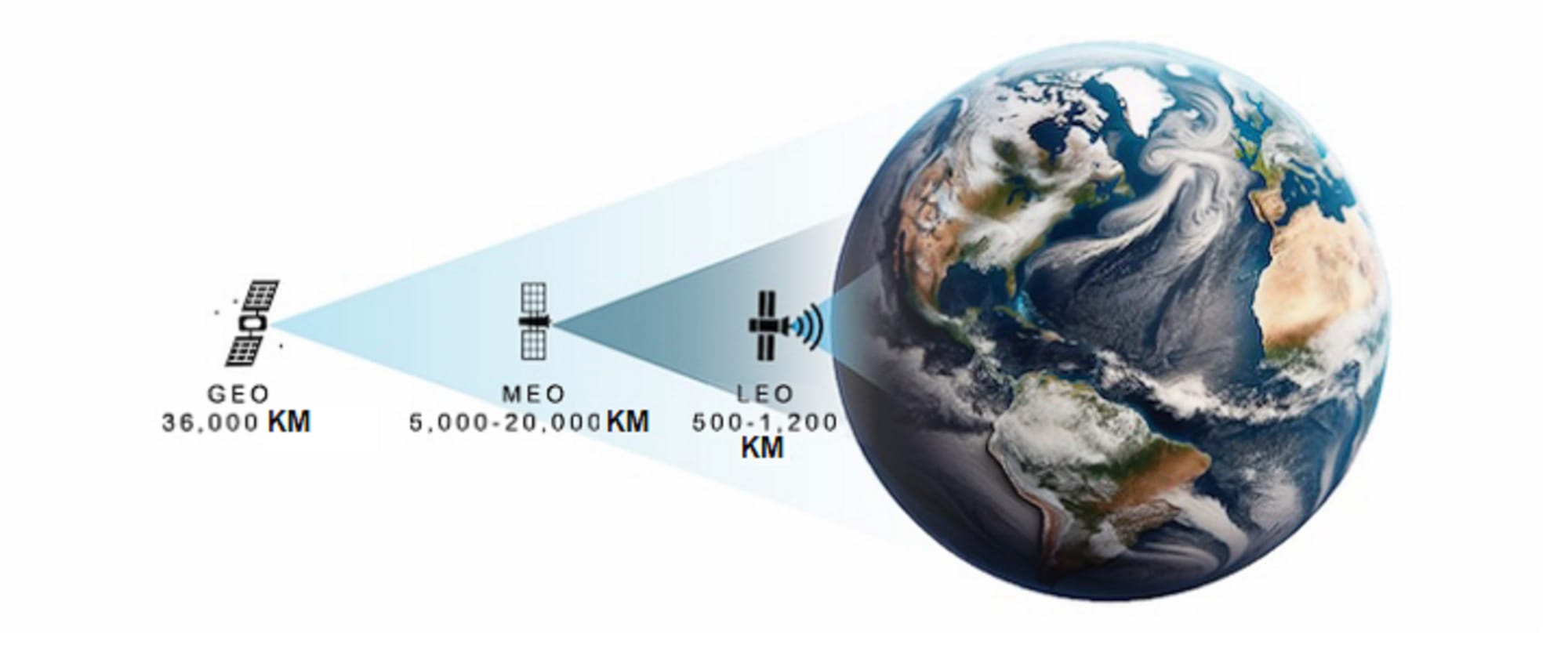
The bottom line: lower orbits mean faster travel, smaller coverage footprints, and lower latency; higher orbits mean slower travel, bigger footprints, and higher latency. This trade-off explains why new constellation architectures often favor swarms of low-flying satellites despite the complexity of managing larger fleets.
Why LEO Needs Constellations
A single satellite in LEO can only cover a fraction of Earth at any moment, and the coverage area moves with the satellite. To achieve continuous global coverage (for worldwide communications or observation), multiple satellites must be spread across different orbital planes.
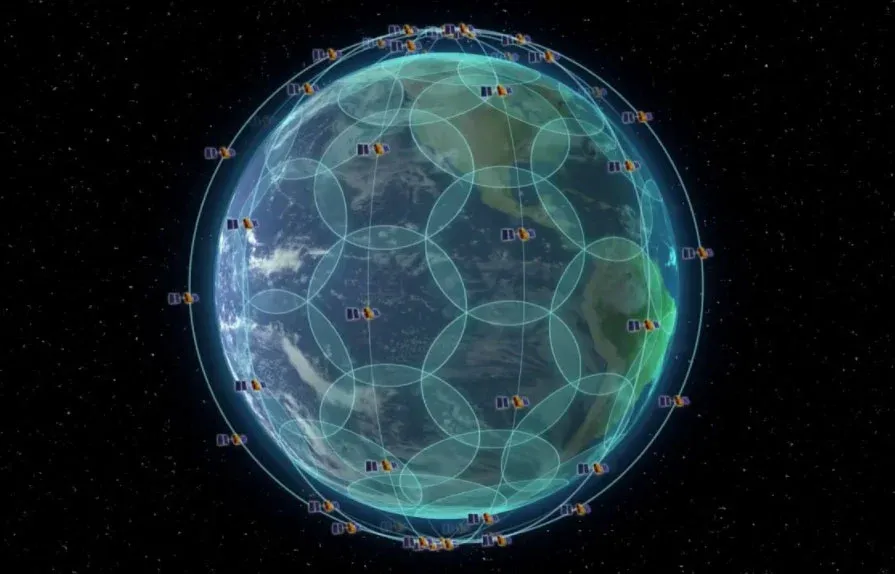
For example, the Iridium communications constellation uses 66 satellites in 6 orbital planes to provide seamless pole-to-pole phone coverage – those 66 satellites work as a team to ensure that as one satellite leaves your area, another comes into view.
Similarly, SpaceX's Starlink internet network deploys thousands of LEO satellites in various "shells" (groups of orbits at different altitudes/inclinations) to create a mesh of coverage. The first Starlink shell alone has 72 orbital planes with 22 satellites each.
In LEO, you typically need dozens of satellites per plane spaced around the globe so that there's always one overhead when and where needed – think of evenly spacing satellites like beads on multiple necklaces encircling Earth. This multi-plane, multi-satellite architecture is mandatory for constant connectivity or observation coverage.
By contrast, GEO satellites, perched high and stationary relative to Earth, can each cover a huge area continuously – one GEO satellite can service an entire continent 24/7. That's why traditional communications systems relied on a few large GEO satellites.
But the downsides of GEO (latency issues and high launch costs per satellite) create opportunities for LEO systems despite needing larger fleets. Understanding orbital mechanics helps investors evaluate the feasibility of constellation business models – effective LEO constellations require significant scale (dozens to thousands of satellites), which drives demand for frequent launches and satellite production capacity.
Satellite Miniaturization and Cost Reduction
From Bus-Sized Behemoths to Toaster-Sized Satellites
Not long ago, satellites were enormous, custom-built machines – satellites like Hubble are literally the size of a school bus. Today, thanks to Moore's Law and electronics miniaturization, we can pack sensors, processors, and radios into satellites as small as a shoebox or even a smartphone.
CubeSats (standardized 10 cm cubes) can be combined to form "toaster-sized" satellites that carry cameras, communication systems, and onboard computers that would impress a 1990s spacecraft engineer. Advances in semiconductor technology, MEMS sensors, and efficient solar panels mean a 5 kg nanosatellite can perform tasks that once required a 500 kg satellite – at a fraction of the cost.
This "smallsat revolution" parallels the personal computer revolution: making space hardware smaller, cheaper, and accessible to far more participants.
Non-Linear Cost Collapse
Crucially, smaller satellites aren't just linearly cheaper – they're orders of magnitude cheaper. A traditional large satellite might cost hundreds of millions (NASA's minivan-sized Lunar Reconnaissance Orbiter cost ~$600M). By contrast, a modern 5-10 kg CubeSat built by a university or startup can cost in the tens or hundreds of thousands of dollars.
In one case, a team of students built a loaf-of-bread-sized CubeSat for under $10,000 in 18 months. Even commercially, a high-end ~100 kg small satellite might cost $1-5 million – still minimal compared to traditional large satellites.
This non-linear drop in cost comes from simplified design, off-the-shelf components, and shorter development cycles. Standardized smallsat platforms and commercially available parts (often leveraging technology from smartphones and laptops) drive costs dramatically lower. Additionally, regulatory and programmatic overhead is reduced – launching a 5 kg satellite doesn't require the multi-agency review boards and decade-long timelines that larger satellites do.
For investors, small satellites dramatically lower the financial and logistical barriers to participate in space, enabling startups, universities, and even smaller companies to deploy orbital assets.
Economics of Many Small Satellites
Small satellites benefit from economies of scale and swarm advantages. Instead of one monolithic satellite, operators can deploy dozens or hundreds of small ones working in concert – often for less total investment.
This approach provides redundancy and resilience: if one satellite fails, the others fill the gap (eliminating single points of failure). It also enables more frequent technology refreshes; Planet Labs, for instance, launches new batches of imaging CubeSats every year or two, iterating rapidly like software updates rather than waiting 15 years to replace a large imaging satellite.
Many small satellites can also outperform a single big satellite in many missions. Daily global imaging is only possible with a fleet of many smallsats (Planet's ~200 Dove satellites image the entire Earth daily), and continuous global internet coverage (like Starlink) requires many small satellites rather than one giant one. And with their close proximity to the earth's surface, their imaging and radio links are greatly improved over large monolithic GEO satellites.
There's a non-linear benefit – ten satellites can do more than ten times what one satellite can do. For instance, adding more satellites to a constellation can exponentially improve revisit time (frequency of coverage), enabling services (like real-time monitoring) that a single satellite could never provide.
Why Small Rockets Matter: FedEx to Space
The Constellation Deployment Challenge
With the shift to smaller satellites deployed in larger numbers, launch requirements have also evolved. Launching one big satellite is like chartering a 747 jet – you fill a large rocket with your payload and go to a specific orbit. But what about launching hundreds of small satellites to dozens of different orbits?
This is the predicament facing modern constellation operators. Rideshare launches on big rockets (like SpaceX Falcon 9 Transporter missions) allow packing many small satellites together, but with a significant limitation: all payloads get dropped off at essentially the same orbit.
The primary customer or rocket operator dictates the destination, and every satellite is released in that general vicinity. If your constellation requires satellites in different orbital planes or specific phasing, your options are limited:
- Use your satellite's onboard propulsion to slowly maneuver to the correct orbit (consuming precious fuel and taking months)
- Wait for a more suitable rideshare opportunity (which may never materialize)
- Book dedicated small launches to place each satellite exactly where needed
As NASA notes, "one of the main disadvantages of rideshare is the inability to get to your desired orbit." This is where small launch vehicles enter the picture – offering dedicated, precise delivery to space.
Responsive & Precise Orbital Delivery
Small rockets (carrying a few hundred kilograms or less) can launch on demand and place satellites exactly where they're needed. Need six satellites each in a different orbital plane to jumpstart your constellation? A Falcon 9 rideshare would place them all in one plane initially, requiring complex maneuvers to redistribute.
Alternatively, a series of dedicated small launches can put each satellite directly into its intended plane and altitude, ready to operate immediately. This precision is increasingly valued by satellite operators who require specific orbits not served by standard rideshares.
Rocket Lab's Electron (lifting approximately 250 kg to LEO) has built its business on this premise – sending small satellites to custom orbits for clients demanding that level of precision. In 2024, Rocket Lab launched the ADRAS-J satellite requiring a very specific trajectory to rendezvous with space debris – something "there is no way you could do on a rideshare."
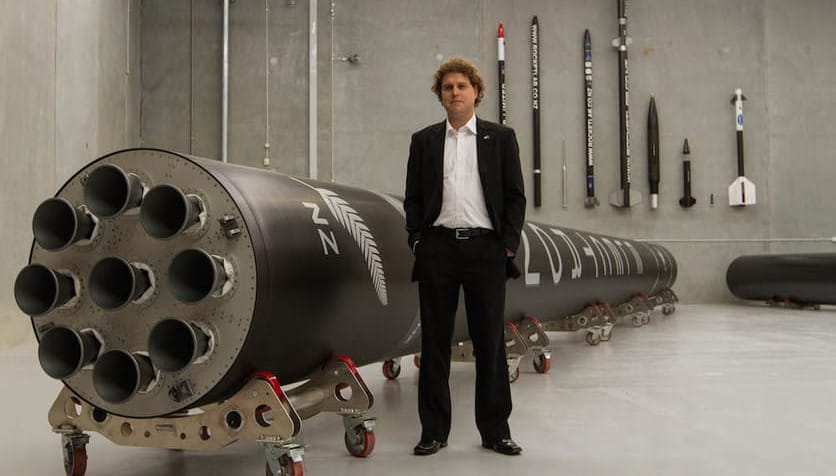
Similarly, if a constellation operator needs a replacement satellite in orbit on a specific date (to fill a gap in coverage), booking a dedicated mini-launch can meet that timeline rather than waiting for the next available rideshare. It's the difference between overnight delivery versus standard shipping.
Rideshare Limitations in Practice
SpaceX's Transporter rideshare missions have dramatically lowered launch costs per kilogram, but they operate like bulk freight service to standardized destinations. Many satellite operators have discovered that this doesn't always meet their needs.
Emerging constellations often "want precise and unique injections" into orbit – at specific inclinations, altitudes, or phasing that standardized rideshare flights cannot accommodate. On a rideshare, you're also tied to the primary mission's schedule; delays to the main payload delay all the secondary ones.
You may also face operational restrictions limiting what your satellite can do until fully separated from others. And there's the challenge of dispersal: all satellites from one launch start clustered together, which is problematic if you want them distributed evenly around an orbital plane.
Some companies employ orbital transfer vehicles (space tugs) to reposition small satellites after a rideshare launch, but this adds cost and complexity to the mission.
The New Wave of Small Launchers
Recognizing these market needs, numerous startups have developed smaller, more agile rockets over the past decade:
- Rocket Lab pioneered this segment with its Electron rocket, completing over 50 launches
- Firefly Aerospace developed the Alpha rocket (~800 kg capacity) for medium-small payloads, completing their first handful of launches
- Vaya Space Developing a novel solid fuel launcher that expects to have their first static tests in the near future
- Phantom Space Developing a cost-effective single-digit millions small satellite launcher that will have static fire tests this year
The appeal is straightforward: if the 20th-century space race emphasized building bigger rockets, the 21st's includes optimizing for efficient delivery of small payloads to specific destinations. Small launchers aim to be the FedEx of space: reliable, responsive, and delivering to the exact orbital address required.
Building a Constellation, One Plane at a Time
To visualize why this matters, consider a company deploying a 60-satellite imaging constellation across 6 orbital planes (10 satellites per plane).
Using rideshares alone, you might launch 10 satellites at a time on large rockets, each batch clustered in a single plane. You'd then need to use the satellites' limited propulsion to gradually move some into other planes – a process that could take months or years for full deployment.
Alternatively, with dedicated small launches, you could dispatch 6 missions, each inserting 10 satellites directly into their targeted planes, achieving full operational capability much faster. Small launch vehicles thus accelerate constellation deployment and enable precise control over the spacing of satellites within their orbits.
This capability is particularly valuable for revisit rate planning – if your constellation needs evenly spaced satellites to observe targets at regular intervals, direct injection into the correct positions saves substantial time and fuel.
Emerging Use Cases Driving Constellation Growth
The demand for small satellite constellations (and consequently, small launch vehicles) is driven by several high-growth applications:
5G Non-Terrestrial Networks
The integration of satellites into 5G networks aims to enable ordinary smartphones to connect via satellite when beyond cellular coverage. This shift from traditional satellite phones requiring bulky specialized handsets to direct smartphone connectivity represents a major market opportunity.
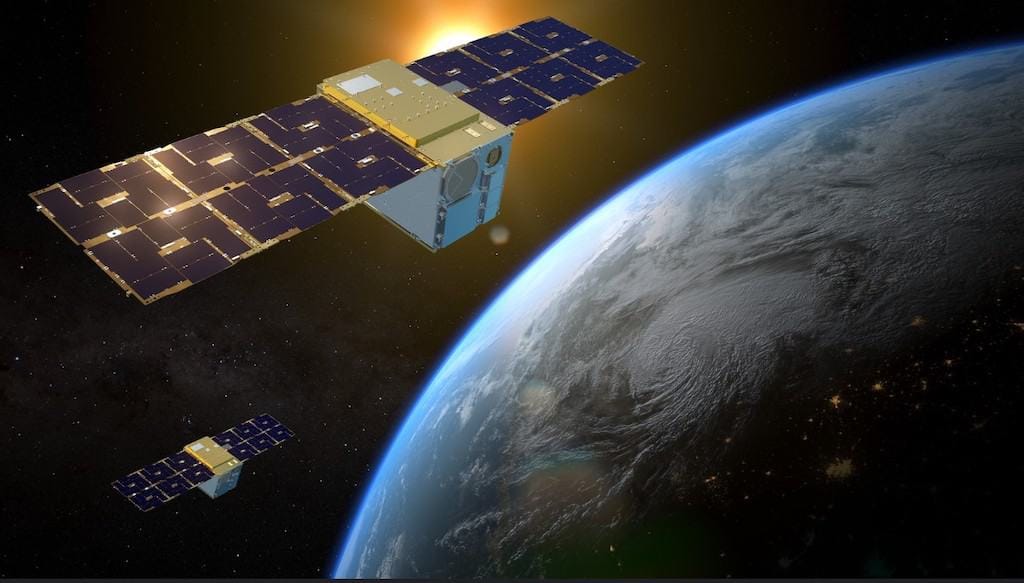
SpaceX and T-Mobile, Apple's emergency satellite messaging, and startups like AST SpaceMobile are all pursuing this vision. The market is projected to reach approximately $30+ billion by 2029, growing at nearly 35% annually – requiring carefully designed multi-plane constellations.
Earth Observation Networks
Modern Earth observation constellations now image the planet daily or hourly in high resolution, serving applications across multiple industries:
- Agriculture: Monitoring global crop health
- Insurance: Assessing disaster damage rapidly and accurately
- Climate monitoring: Tracking environmental changes
- Urban development: Observing infrastructure growth
- Defense and intelligence: Providing near-real-time imagery of global hotspots
These observation networks require satellites distributed in precise orbital arrangements to achieve optimal coverage and revisit rates – a perfect use case for dedicated small launch capabilities.
IoT Infrastructure in Space
The Internet of Things requires connectivity in remote locations beyond terrestrial networks. Satellite IoT constellations connect millions of sensors, trackers, and devices worldwide, typically using small satellites to relay modest data packets.
The satellite IoT sector is projected to grow from approximately $1 billion in 2023 to $5-6 billion by 2030, with applications spanning agriculture, maritime shipping, energy infrastructure, and environmental monitoring. Each application benefits from precisely deployed satellites ensuring consistent global coverage.
Persistent Intelligence, Surveillance, and Reconnaissance
Both government and commercial operators are deploying satellite networks for continuous monitoring capabilities. The U.S. National Reconnaissance Office has shifted to a "proliferated architecture" of numerous smaller satellites across multiple orbits to deliver substantially more data with faster revisit times.
Commercial companies like BlackSky, Capella Space, and HawkEye 360 now offer near-real-time imagery and RF monitoring services. During the Ukraine conflict, BlackSky demonstrated its agility by deploying satellites to new orbits for improved coverage – showcasing the flexibility enabled by responsive small launch capabilities.
Investment Implications
For investors evaluating the space sector, understanding the interrelationship between rockets, orbits, and satellites provides crucial context. Several key trends emerge from this technical foundation:
- Satellite miniaturization is driving a non-linear decrease in spacecraft costs while maintaining or improving capabilities, opening space to more participants
- Constellation architectures (many small satellites vs. few large ones) offer advantages in resilience, refresh rate, and certain performance metrics
- Orbit selection involves fundamental trade-offs in coverage, latency, and cost that directly impact business models
- Launch strategies must balance cost, precision, and timing considerations to optimize constellation deployment
Small launch vehicles represent a distinct segment with a value proposition separate from heavy lift providers. Rather than competing directly with SpaceX or other established large rocket companies, successful small launchers target this growing market of small satellite constellations:
- Orbit precision specialists – Delivering satellites to exact parameters not served by standard rideshares
- Responsive launch providers – Offering rapid deployment for time-sensitive missions
- Constellation deployment enablers – Facilitating efficient distribution across multiple planes
- National-level launch capacity – Providing sovereign access to space
When assessing small launcher investments, key factors include manufacturing efficiency, launch cadence capabilities, reliability record, customer diversification, and path to profitability. The ongoing constellation boom creates persistent and growing demand for precision orbital delivery.
The Launch Landscape Ahead
Rather than a winner-take-all scenario, the space transportation sector is diversifying to address varied mission requirements – similar to how the aviation industry supports everything from jumbo jets to specialized helicopters.
Small launchers fill a crucial and rapidly growing need in this evolving landscape, enabling the distributed architectures driving the current space renaissance. When evaluating satellite ventures, investors should carefully assess the launch strategy – a constellation reliant solely on rideshares may face deployment delays and orbital compromises, whereas one budgeting for dedicated launches can mitigate those risks.
As Space 3.0 unfolds with thousands of satellites creating new orbital infrastructure, small launchers will remain essential components of a functioning space economy. The physics of orbital mechanics and the business case for precision deployment ensure small launch will continue as a distinct and valuable segment of the space transportation market – not because small is inherently better, but because sometimes the most efficient solution isn't the biggest rocket, but the right-sized one for the mission at hand.